
A Study on hydrogen dispersion characteristics according to the length of the exhaust duct in the fuel gas supply system room
Copyright © The Korean Society of Marine Engineering
This is an Open Access article distributed under the terms of the Creative Commons Attribution Non-Commercial License (http://creativecommons.org/licenses/by-nc/3.0), which permits unrestricted non-commercial use, distribution, and reproduction in any medium, provided the original work is properly cited.
Abstract
Hydrogen has wide-ranging flammability, lower minimum ignition energy, and higher flame propagation speed than hydrocarbon-based fuels. If a leak accident occurs on a ship that uses hydrogen as fuel, there is a high possibility that it will lead to an explosion or fire. A confined space, such as a fuel-gas supply system room on a ship, should be provided with effective mechanical ventilation systems to prevent the accumulation of hydrogen. An exhaust duct must also be installed to prevent the discharged gas from spreading to hazardous areas. In this study, the dispersion characteristics of hydrogen according to the length of the exhaust duct in a fuel–gas supply system were numerically investigated. The FLACS program was used for numerical analysis, and the numerical analysis technique was compared and verified with the experimental results. Numerical analysis confirmed that the exhaust duct length affected the dispersion characteristics inside the fuel gas supply system room. The numerical analysis results of this study are expected to be useful in the design and installation of ventilation systems and exhaust ducts.
Keywords:
Hydrogen gas, Fuel gas supply system room, Ventilation system, Exhaust duct, FLACS1. Introduction
Hydrogen, which is renewable and eco-friendly, is considered the most promising energy source for overcoming fossil fuel depletion and air pollution problems [1][2][3]. Hydrocarbon-based fuels have lower minimum ignition energy and a wide-ranging flammability limit than hydrocarbon-based fuels.
Therefore, the associated hazards must be evaluated before hydrogen can be safely used as fuel. In the event of a hydrogen gas leak, the density of hydrogen is lower than that of air, which causes hydrogen to disperse into the atmosphere in open spaces. However, the situation becomes hazardous in enclosed spaces such as ships' storage and engine rooms because the less dense hydrogen gas cloud stagnates and accumulates near the ceiling [4]. An accumulated hydrogen gas cloud poses a high risk of fire or explosion, even with a minor ignition source.
The wide range of hydrogen flammability limits (4–75%) [5][6] requires investigation of the mitigation system to maintain the concentration within safety limits. Many studies have been conducted on hydrogen dispersion in semiconfined enclosures [5][7]-[12]. The concentration levels in the enclosure mainly depend on the hydrogen leakage source (mass flow rate, pressure, location, and direction) and the ventilation area [8][10][11]. Insufficient ventilation results in higher concentrations and requires a longer time to reduce them under the flammability limit [10].
Merilo et al. [9] investigated the risk of deflagration in a private garage caused by leakage from a car. The concentration ranged from a mass flow rate of 1.52 kg/h to 9.22 kg/h, resulting in well-mixed layers beneath the ceiling (with both natural and mechanical ventilation). The findings indicated a reduction in the concentration as the ventilation rates increased. Experiments using the highest mass flow rates of 4.92 kg/h and 6.7 kg/h, coupled with the lowest ventilation rate, exhibited an average concentration (at ignition time) exceeding 10%, escalating deflagration hazards.
Hussein et al. [13] numerically investigated hydrogen release in a naturally ventilated enclosure was performed by Hussein et al. [13]. This study investigated hydrogen concentrations resulting from blowdown-type releases at 700 bar using Thermal and Pressure Relief Devices (TPRDs) diameters. The release source was positioned between the rear wheels beneath the car. The TPRDs with diameters larger than 0.5 mm generated a flammable cloud that filled most of the enclosure within 20 s.
The authors highlighted the issue of excessively large TPRD diameters, which led to rapid high-concentration buildups, potentially causing the pressure-peaking phenomenon observed in earlier studies referenced in this article [14]-[17]. Malakhov et al. [18] explored forced ventilation as a mitigation method in semi-enclosed spaces. Using computational fluid dynamics (CFD) and experiments, they examined the concentration distribution resulting from the horizontal hydrogen release. Their findings illustrated the impact of mechanical ventilation on the hydrogen jet behavior, its length, and the reduction in hydrogen concentration within the tested compartment.
Park et al. [19] investigated the effect of explosion load characteristics in varying ventilation tunnel geometrics to design systems for hydrogen ship compartments. Also, experiments were conducted to investigate the impact of the tunnel length through which the explosion wave was transmitted during an explosion to determine the explosion characteristics and maximum pressure. The International Maritime Organization code recommends installing ventilation devices in confined spaces to prevent gas accumulation [20]. In addition, installation guidelines were provided for the exhaust duct length.
The exhaust duct length is an important variable when selecting a mechanical ventilation system that meets the ventilation standards for the fuel-supply preparation room. The longer the exhaust duct, the more friction loss that occurs inside the exhaust duct, which reduces the performance of the mechanical ventilation system. Also, it can be confirmed from Park's study [19] that overpressure varies depending on the exhaust duct length when an explosion occurs inside the exhaust duct.
Therefore, it is necessary to identify hydrogen dispersion characteristics and ventilation performance based on changes in the exhaust duct lengths in the fuel gas supply system room. This study conducted numerical investigations on the dispersion characteristics of hydrogen under different exhaust duct lengths. The numerical analysis technique was verified and compared with the experimental results. The numerical analysis results of this study are expected to be useful for designing ventilation systems and establishing ventilation-related regulations.
2. Hydrogen Dispersion Experiment
A hydrogen gas dispersion experiment was performed to verify the FLACS modelling technique [21].
2.1 Experimental Setup
The dispersion experiment was conducted in a 10.2 m × 5.3 m × 2.65m enclosure, representing a ship's fuel gas supply system room. It was lined with vinyl to prevent hydrogen leakage elsewhere in the enclosure, as shown in Figure 1.
This study implemented leakage conditions using hydrogen gas supply equipment and control systems, releasing hydrogen through a flat nozzle with a diameter of 4 mm(Figure 2). The leak rate was 900 LPM(0.001257 kg/s) at 5 bar, and the leak duration was set to 300 s. If a leak occurs in a high-pressure hydrogen pipe, it is expected to occur in amounts exceeding 900 LPM; the experiment was conducted at the maximum flow rate of the MFC used in the experiment. In addition, the leakage period was assumed to be 300 s, which is the time until all safety facilities, such as the ESD valves, are fully operational. The leak location was one point at the center of the room floor of the fuel gas supply system.
Exhaust ducts were installed at the corners of the ceiling. One was 1.5m long and used for air intake; the other was 5m long, and a forced ventilation fan was installed (Figure 3).
The ventilation capacity was calculated based on the volume of the experimental facility to satisfy at least 30 air changes per hour [20]. The forced ventilation fan began operating immediately after the leakage ceased. In the experiment, the exhaust fan was operated at its maximum performance, and the wind speed in the exhaust duct was measured to check the performance of the exhaust fan, and the value was 4.5 m/s.
The oxygen concentration in the enclosure was measured using an optical sensor. Existing hydrogen sensors can measure only when the surrounding mixed gas touches the contact surface of the sensor; therefore, they only measure the approximate concentration around the contact surface of the sensor rather than the exact gas concentration. However, the optical oxygen sensor used in this study is a flow-through-type sensor with the advantage of measuring the exact gas concentration at the desired point because it measures the sampling gas through a tube. Optical oxygen sensors were installed at eight points to measure the hydrogen concentration inside the fuel supply preparation room. Figure 4 shows the location and installation of the sensors.
Figure 5 shows the hydrogen concentrations over time as measured by the sensors at a leak rate of 900 LPM. The hydrogen concentration at point MP4 above the nozzle was the highest, whereas, at MP2, the hydrogen concentration was the lowest.
3. Numerical Analysis of Dispersion
This section describes numerical modelling techniques using the FLACS program, which is a CFD tool. The numerical analysis results were compared and verified with the dispersion experimental results, and a dispersion analysis for various exhaust duct lengths was performed to determine the hydrogen concentration and ventilation performance according to the exhaust duct length.
3.1 Numerical Modelling
The fuel gas supply system room used in the experiment was modeled, as shown in Figure 6. In the dispersion simulations, FLACS was sensitive to mesh size. Therefore, the grid recommendations in FLACS were followed [19]: grid size of the core domain = 0.1 m and total number of cells ≈ 129,900.
3.2 Verification of Numerical Modelling
The same conditions as in the experiment were used to verify the numerical modelling technique.
The exhaust conditions were not implemented by applying the performance of the ventilation fan, such as the exhaust air volume, but by the speed inside the exhaust duct measured in the experiment.
Figure 7 and Table 1 compare the experimentally measured and FLACS-simulated hydrogen concentrations over time at each location. As shown in Figure 7, the maximum concentration was approximately 2% higher in the experimental results than in the numerical analysis. The difference between the experimental and numerical analysis results is that although the nozzle used in the experiment was fan-shaped, implementing a fan-shaped nozzle in the numerical analysis took a lot of work.
3.3 Simulation of Hydrogen Gas Dispersion under Various Exhaust Duct Lengths
This section discusses the effects of the exhaust duct length in a confined space. As confirmed in Section 3.3, FLACS is a reliable tool for estimating maximum hydrogen concentration and ventilation performance.
Figure 9 illustrates the numerical model of FLACS depicting different exhaust duct lengths. In this study, a gas dispersion simulation was performed by changing the exhaust duct lengths to 1m, 3m, and 5m, and the same leakage and exhaust conditions were applied. In the case of exhaust conditions, there is a limitation in that the performance of the exhaust fan was not accurately measured in the experiment, so in the numerical analysis, the mass flow rate(0.716 kg/s) was calculated based on exactly 30 ventilations per hour and the exhaust conditions were applied.
Figure 10 shows the hydrogen concentration over time for various exhaust duct lengths at each location, and Figure 11 shows the hydrogen concentration distribution at 300s. Table 2 summarizes the maximum concentration and ventilation performances of the simulations.
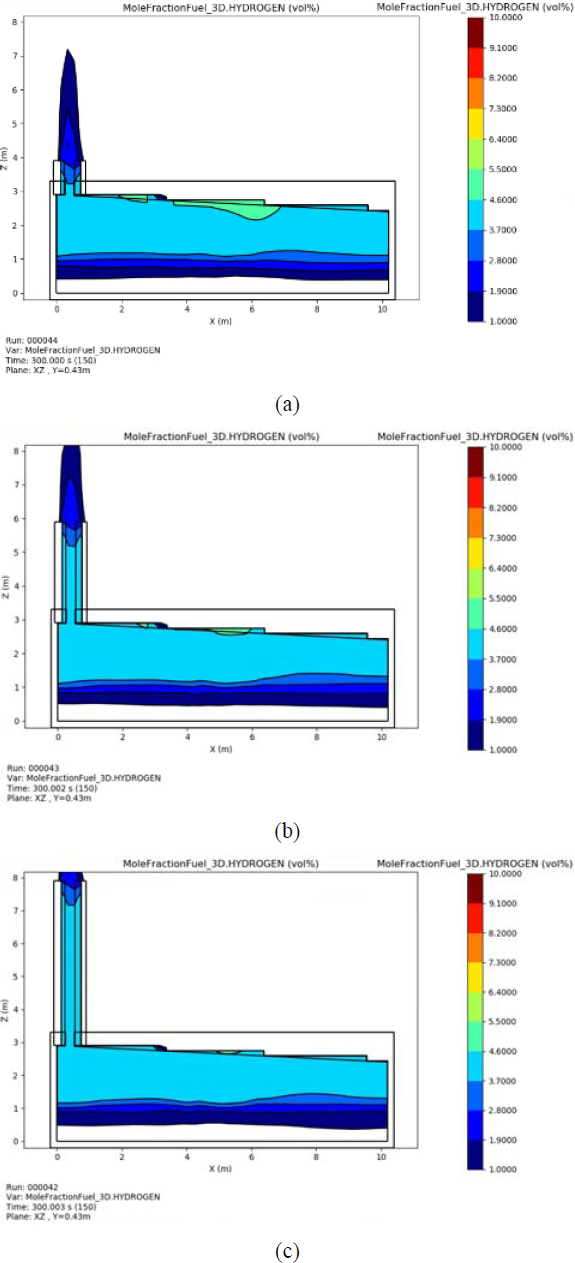
Hydrogen concentration distribution according to exhaust duct length at 300s: (a) 1m; (b) 3m; (c) 5m
In Figures 10 and 11, it can be observed that shorter exhaust duct lengths correlate with higher hydrogen concentrations. Until the ventilation fan started operating, hydrogen gas entered the exhaust duct, causing a decrease in the hydrogen concentration at the monitoring points.
In the case of ventilation performance, it can be seen that the case with a 5m exhaust duct length and a low maximum concentration takes a short time to reach 25% of the LFL.
Figure 12 shows the velocity results at the starting point of the exhaust duct (MP 6). Numerical analysis showed that the speed inside the exhaust duct increased because the same mass flow rate was applied to the end of the exhaust duct.
Figure 13 illustrates that the flammable volume (m3) within the fuel gas supply system room, excluding the exhaust duct, was the highest when the exhaust duct length was 1 m.
4. Conclusion
Hydrogen leaks from confined spaces such as a ship's storage or engine room and presents a significant hazard to potential fires or explosions, even in the presence of a minor ignition source.
Consequently, installing a ventilation system is imperative to mitigate this risk. This study numerically investigated the dispersion characteristics about hydrogen gas clouds and the efficacy of ventilation systems in enclosed spaces with diverse exhaust duct lengths. Various exhaust duct lengths were subjected to numerical analysis after comparing and validating the FLACS-modeled technique against the experimental results. The conclusions and observations are as follows.
- 1. Validation of numerical analysis: The numerical analysis ascertained that while the hydrogen concentration and ventilation performance exhibited a trend consistent with the experimental results, there were notable disparities between them. These disparities are attributed to the inherent challenges in modelling uncontrollable factors, including, but not limited to, air circulation, air resistance, hydrogen-oxygen production ratio, and the permeability of air and hydrogen. Additionally, it is imperative to note that the experimental nozzle employed could not be directly implemented in FLACS. Despite these challenges, the overall alignment of trends suggests that FLACS is a dependable tool for designing ventilation systems. However, further research is essential to refine its accuracy and applicability.
- 2. Effect of exhaust duct length: The numerical analysis results confirmed that the exhaust duct length did not significantly affect the ventilation performance. Under identical leak conditions, shorter exhaust duct lengths resulted in higher concentrations within the fuel supply preparation room, primarily because the hydrogen migrated into the exhaust duct. Regarding ventilation performance, the 5m exhaust duct length proved superior by 8–43s compared to the 1m exhaust duct length. However, additional experiments are required to verify these results.
The data collected in this study are expected to be instrumental in designing ventilation layouts for hydrogen facilities. Furthermore, the findings of this numerical analysis provide valuable insights into incorporating hydrogen fuel in compliance with the IGF Code. These insights may prove valuable for enhancing ventilation systems in the maritime domain.
Acknowledgments
This work was supported by the Technology development Program(RS-2023-00223011) funded by the Ministry of SMEs and Startups(MSS, Korea).
Author Contributions
Conceptualization, J. H. Kim. and J. K. Seo.; Methodology, J. H. Kim and H. J. Kim; Software, J. H. Kim and H. J. Kim; Validation, J. H. Kim and S. W. Park; Formal Analysis, J. H. Kim and J. K. Seo; Investigation, J. H. Kim; Resources, J. H. Kim; Data Curation, J. H. Kim and S. W. Park; Writing—Original Draft Preparation, J. H. Kim and J. K. Seo; Writing—Review & Editing, J. H. Kim and J. K. Seo; Visualization, H. J. Kim; Supervision, J. H. Kim and J. K. Seo; Project Administration, J. H. Kim and H. J. Kim; Funding Acquisition, H. J. Kim.
References
-
J. O. Abe, A. P. I. Popoola, E. Ajenifuja, and O. M. Popoola, “Hydrogen energy, economy and storage: Review and recommendation,” International Journal of Hydrogen Energy, vol. 44, no. 29, pp. 15072-15086, 2019.
[https://doi.org/10.1016/j.ijhydene.2019.04.068]
-
I. Staffell, D. Scamman, A. V. Abad, P. Balcombe, P.E. Dodds, P. Ekins, N. Shah, and K. R. Ward, “The role of hydrogen and fuel cells in the global energy system,” Energy & Environmental Science, vol. 12, no. 2, pp. 463-491, 2019.
[https://doi.org/10.1039/C8EE01157E]
-
D. Gielen, F. Boshell, D. Saygin, M. D. Bazilian, N. Wagner, and R. Gorini, “The role of renewable energy in the global energy transformation,” Energy Strategy Reviews, vol. 24, pp. 35-50, 2019.
[https://doi.org/10.1016/j.esr.2019.01.006]
- S. J. Kim, H. H. Lee, S. W. Park. D. Y. Baeg, J. H. Kim, and J. K. Seo, “Blast loading profile of gaseous hydrogen in a confined space under various leak conditions,” International Conference on Ocean, Offshore, and Arctic Engineering (OMAE) proceedings, 2022.
-
M. Dadashzadeh, A. Ahmad, and F. Khan, “Dispersion modelling and analysis of hydrogen fuel gas released in an enclosed area: A CFD-based approach,” Fuel, vol. 184, pp. 192-201, 2016.
[https://doi.org/10.1016/j.fuel.2016.07.008]
-
M. De Stefano, X. Rocourt, I. Sochet, and N. Daudey, “Hydrogen dispersion in a closed environment,” International Journal of Hydrogen Energy, vol. 44, no. 17, pp. 9031-9040, 2019.
[https://doi.org/10.1016/j.ijhydene.2018.06.099]
-
J. He, E. Kokgil, L. L. Wang, and H. D. Ng, “Assessment of similarity relations using helium for prediction of hydrogen dispersion and safety in an enclosure,” International Journal of Hydrogen Energy, vol. 41, no. 34, pp. 15388-15398, 2016.
[https://doi.org/10.1016/j.ijhydene.2016.07.033]
-
W. M. Pitts, J. C. Yang, and M. G. Fernandez, “Helium dispersion following release in a 1/4-scale two-car residential garage,” International Journal of Hydrogen Energy, vol. 37, no. 6, pp. 5286-5298, 2012.
[https://doi.org/10.1016/j.ijhydene.2011.12.008]
-
E. G. Merilo, M. A. Groethe, J. D. Colton, and S. Chiba, “Experimental study of hydrogen release accidents in a vehicle garage,” International Journal of Hydrogen Energy, vol. 36, no. 3, pp. 2436-2444, 2011.
[https://doi.org/10.1016/j.ijhydene.2010.04.056]
-
S. Gupta, J. Brinster, E. Studer, and I. Tkatschenko, “Hydrogen related risks within a private garage: Concentration measurements in a realistic full scale experimental facility,” International Journal of Hydrogen Energy, vol. 34, no. 14, pp. 5902-5911, 2009.
[https://doi.org/10.1016/j.ijhydene.2009.03.026]
-
B. Cariteau, J. Brinster, E. Studer, I. Tkatschenko, and G. Joncquet, “Experimental results on the dispersion of buoyant gas in a full scale garage from a complex source,” International Journal of Hydrogen Energy, vol. 36, no. 3, pp. 2489-2496, 2011.
[https://doi.org/10.1016/j.ijhydene.2010.04.053]
-
T. Beard, M. Bragin, W. Malalasekera, and S. S. Ibrahim, “Numerical simulation of hydrogen discharge in a partially enclosed space,” Energy Procedia, vol. 66, pp. 153-156, 2015.
[https://doi.org/10.1016/j.egypro.2015.02.083]
-
H. Hussein, S. Brennan, and V. Molkov, “Dispersion of hydrogen release in a naturally ventilated covered car park,” International Journal of Hydrogen Energy, vol. 45, no. 43, pp. 23882-23897, 2020.
[https://doi.org/10.1016/j.ijhydene.2020.06.194]
-
S. Brennan and V. Molkov, “Pressure peaking phenomenon for indoor hydrogen releases,” International Journal of Hydrogen Energy, vol. 43, no.39, pp. 18530-18541, 2018.
[https://doi.org/10.1016/j.ijhydene.2018.08.096]
-
D. Makarov, V. Shentsov, M. Kuznetsov, and V. Molkov, “Pressure peaking phenomenon: Model validation against unignited release and jet fire experiments,” International Journal of Hydrogen Energy, vol. 43, no. 19, pp. 9454-9469, 2018.
[https://doi.org/10.1016/j.ijhydene.2018.03.162]
-
A. W. Lach, A. V. Gaathaug, and K. Vaagsaether, “Pressure peaking phenomena: Unignited hydrogen releases in confined spaces Large scale experiments,” International Journal of Hydrogen Energy, vol. 45, no. 56, pp. 32702-32712, 2020.
[https://doi.org/10.1016/j.ijhydene.2020.08.221]
-
A. W. Lach and A. V. Gaathaug, “Large scale experiments and model validation of pressure peaking phenomenaignited hydrogen releases,” International Journal of Hydrogen Energy, vol. 46, no. 11, pp. 8317-8328, 2021.
[https://doi.org/10.1016/j.ijhydene.2020.12.015]
-
A. A. Malakhov, A. V. Avdeenkov, M. H. du Toit, and D. G. Bessarabov, “CFD simulation and experimental study of a hydrogen leak in a semi-closed space with the purpose of risk mitigation,” International Journal of Hydrogen Energy, vol. 45, no. 15, pp. 9231-9240, 2020.
[https://doi.org/10.1016/j.ijhydene.2020.01.035]
-
S. W. Park, J. H. Kim, and J. K. Seo, “Explosion characteristics of hydrogen gas in varying ship ventilation tunnel geometries: An experimental study,” Journal of Maine Science and Engineering, vol. 10, no. 4, p. 532, 2022.
[https://doi.org/10.3390/jmse10040532]
- International Maritime Organization(IMO), International Code of Safety for Ships Using Gases or Other Lowf-lashpoint Fuels, 2015.
- FLACS-CFD v21.2, User’s Manual, 2023.